Entry Version:
Citation:
Pancreapedia: Exocrine Pancreas Knowledge Base, DOI: 10.3998/panc.2012.13
Attachment | Size |
---|---|
![]() | 865.93 KB |
Gene Symbols:
1. General Information
PKC isoforms and activators
The protein kinase C (PKC) family of serine/threonine kinases, is composed of 10 isoforms which are divided into three classes: conventional (α, ß1, ßII, γ), novel (δ, ε, θ, η) and atypical (ξ, ι/Λ) (Figure 1). Other isoforms, PKC μ and ν, have since been reclassified as a distinct family called protein kinase D (PKD). All PKC isoforms share a conserved kinase domain, however, they have differences in their regulatory sites. The regulatory domains are defined by a pseudosubstrate (autoinhibitory) region and one or two membrane-targeting modules (C1 and C2 domains). Conventional PKC (cPKC) are activated by diacylglycerol (DAG) and calcium, which binds to C1 and C2, respectively (39, 78). The novel PKCs (nPKC) are activated by DAG and the atypical PKCs (aPKC) are activated by mechanisms involving phosphoinositides and phosphorylation (42). In addition, DAG-sensitive PKC isoforms can be activated pharmacologically using phorbol esters (Table 1) (47, 55).
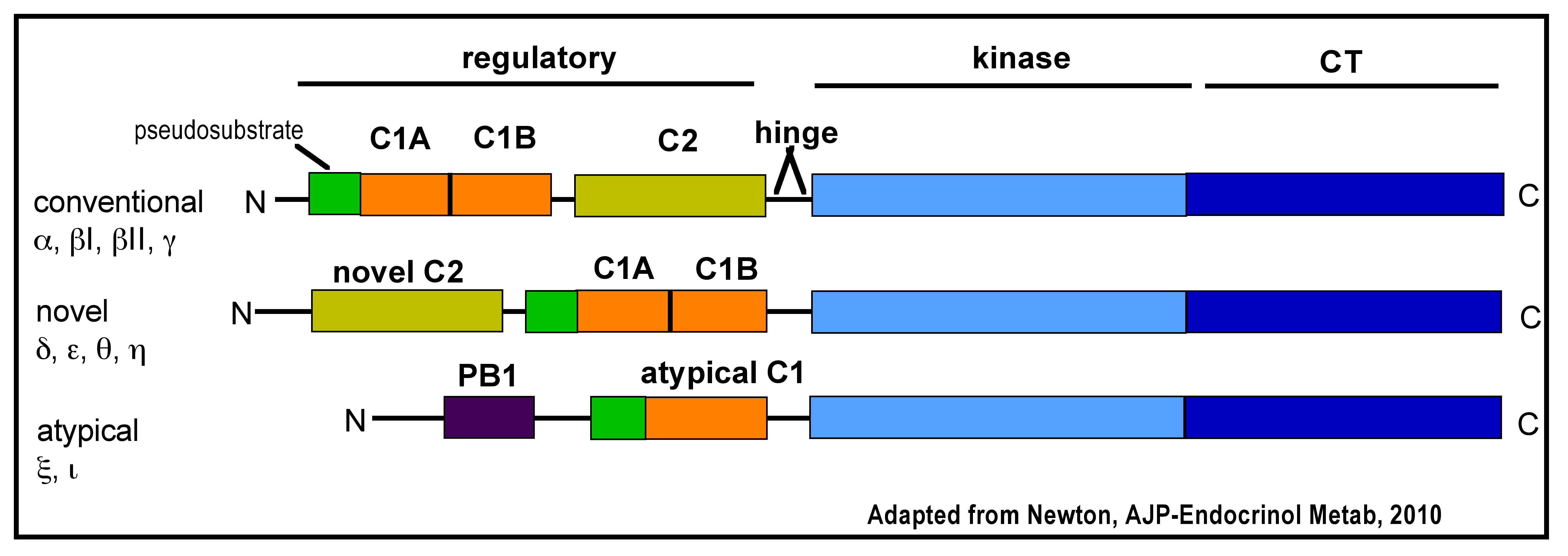
Figure 1. PKC Family. Domain composition of PKC isoforms: pseudosubstrate (green rectangle), C1 domain [orange rectangle; C1B domain binds diacylglycerol (DAG)], C2 domain [yellow rectangle; Ca2+ binding], hinge segment, kinase domain (light blue) and carboxyl-terminal tail (CT; dark blue rectangle).
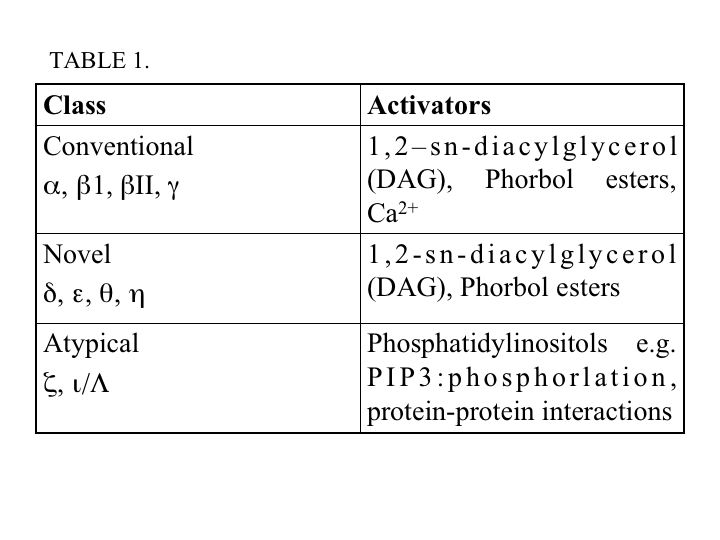
Maturation
Binding of heat shock protein-90 (HSP-90) and the mammalian target of rapamycin complex 2 (mTORC2) are important for the phosphorylation-dependent maturation of nascent PKC, particularly cPKC and nPKC, (Figure 2A, B). PKC isoforms share three phosphorylation sites located in the kinase domain and in the carboxy-terminal tail. These phosphorylation sites are in the activation loop, and the turn and hydrophobic motifs, which are important for attaining catalytic competence and stability. The activation loop is phosphorylated by the upstream kinase phosphoinositide-dependent kinase-1 (PDK-1), either constitutively for nPKC and cPKC or by an agonist for aPKC (Figure 2A). However, unlike other isoforms, PKCδ does not require activation loop phosphorylation to attain catalytic competence. Phosphorylation of the turn motif depends on mTORC2 but it is unknown if mTORC2 is directly or indirectly involved (Figure 2B). The mechanism of phosphorylation for the hydrophobic motif is unclear in intact cells, however, kinetic analyses indicates that the hydrophobic motif is autophosphorylated by an intramolecular reaction. Phosphorylation of these sites is also important for attaining maximal enzyme activities (39).
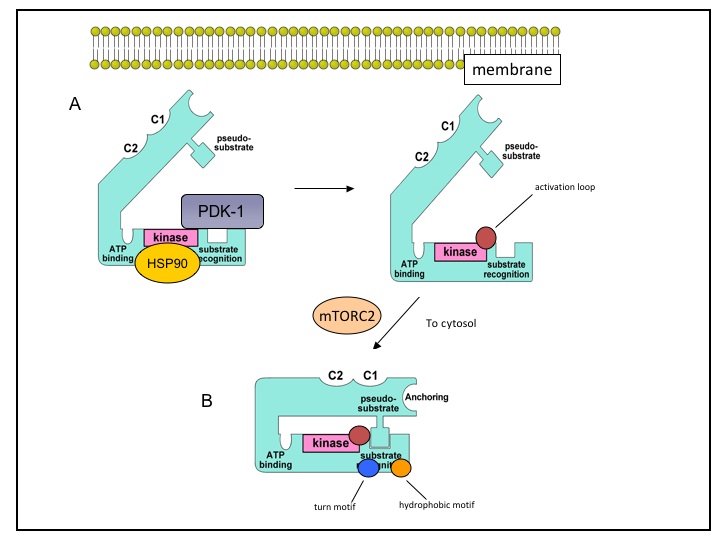
Figure 2. PKC maturation. Nascent PKC is found in an inactive open conformation associated with membrane fractions. PKC must first undergo maturation through a series of phosphorylations to attain catalytic competence. HSP90 binds in the kinase domain while PDK-1 binds in the carboxy-terminus and phosphorylates the activation loop (red circle; 2A). Following activation loop phosphorylation, mTORC2 is involved in phosphorylations of the turn motif (blue circle) and the hydrophobic motif (orange circle). The fully mature enzyme is localized to the cytosol with the pseudosubstrate blocking the substrate-binding pocket (2B).
Translocation and activation
PKC activation requires two distinct events: association of PKC with activators and exposure of the catalytic regions of the enzyme required to phosphorylate its substrates. PKC is held in the inactive state by the binding of an internal pseudosubstrate region to its substrate-binding site (Figure 3A). PKC activation is usually initiated by translocation of the enzyme to its target site where it becomes tightly associated with membranes (Figure 3B) (39). Experiments have shown that PKC translocation is not inhibited by depolymerization of filamentous actin or tubulin, suggesting that neither cytoskeletal protein is involved in PKC translocation. Further, photobleaching experiments have shown that PKC freely diffuses in the cytosol (57). Lipid hydrolysis is involved in the recruitment of PKC isoforms to membranes by Ca2+ and/or DAG. Binding of Ca2+ to cPKC’s C2 domain pre-targets the enzyme to the plasma membrane where it binds phosphatidylinositol-4,5-bisphosphate (PIP2), phosphatidyl serine (PS) and DAG, thus promoting dissociation of the pseudosubstrate from the substrate-recognition domain (FIGURE 3B). This exposes PKC’s ATP-binding site and kinase domain, and allows binding of the target protein (substrate) and its subsequent phosphorylation (Figure 3C, D). For nPKC, recruitment to the plasma membrane involves DAG. The C1 domain of nPKC has an intrinsic high-affinity for DAG that appears sufficient to target it to membranes upon agonist-stimulated increases in DAG. The nPKCs also have a high basal localization to membranes enriched in DAG such as the Golgi (39). However, atypical isoforms do not bind DAG or Ca2+, rather phosphotidylinositol-3,4,5-triphosphate (PIP3) and other lipids appear to mediate its translocation and activation. Though it remains unclear how PIP3 activates aPKC, two mechanisms have been proposed for activation of the aPKCξ isoform: directly by binding of PIP3 to the PKCξ pleckstrin homology (PH) domain or an indirect effect mediated by a PIP3/PDK complex (40). Other studies indicate that aPKC is allosterically activated by an interaction of its Phox/Bem1 (PB1) domain with a partitioning defective 6 (PAR6)-CDC42 complex (54).
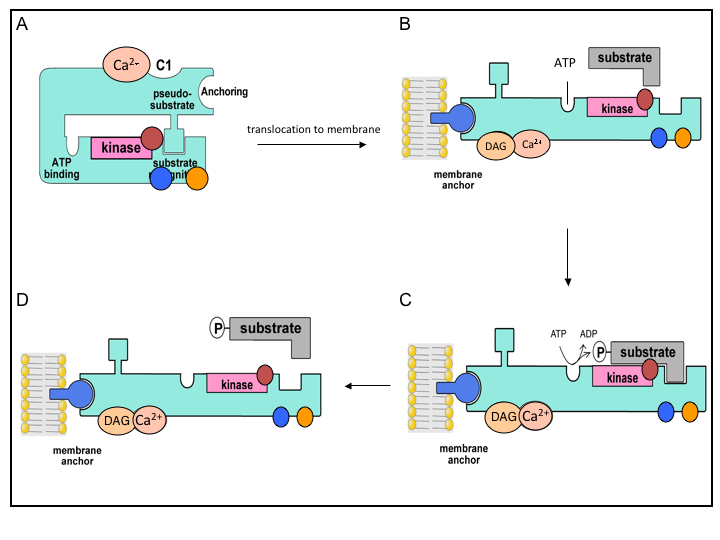
Figure 3. Model of conventional PKC Activation. In the inactive state (A), the PKC isozyme is in a “closed” conformation with the pseudosubstrate blocking the substrate binding region. Following agonist stimulated lipid hydrolysis, PKC is activated through a series of sequential activation steps: PKC binds Ca2+ (A) and translocates to membranes where binding of DAG occurs and promotes the activation and opening of the isozyme (B). The activated isozyme can now bind ATP and phosphorylate various substrates (C, D). Phosphorylations: activation loop (red circle), turn motif (blue circle), hydrophobic motif (orange circle).
Membrane localization
The tight association of PKCs with membranes appears to be mediated by their binding to membrane lipids and as well to specific membrane proteins known as, the “receptors for activated C kinase” or RACKs (39, 52). This family of membrane proteins binds active, phosphorylated PKC. Since RACKS are localized to specific subcellular compartments, they are an important mechanism for targeting PKC (14). RACKs are also believed to bring PKC into close proximity with its substrate, thereby allowing functional specificity for different isoforms. PKC binding to RACKs is isoform specific and occurs after the first step in PKC activation. Isoform specific inhibitors have been developed through an understanding of the PKC interaction with RACKs. These isoform specific translocation inhibitors, which have been developed for PKCδ and -ε, are peptides that correspond to the RACK-binding site of PKC (14). Peptides, known as pseudo-RACK peptides, have also been identified which induce binding of PKC to its RACK in the absence of second messenger activation (53). These pseudo-RACKs stabilize PKC for binding with RACK and act as activators in vitro (53, 58). Other scaffold proteins have also been shown to bind PKC in its various conformations (“non-phosphorylated”, “inactive but phosphorylated”, “active and dephosphorylated”) and are critical for the regulation and function of PKC(39).
This section has summarized the mechanisms of PKC maturation, translocation and activation. The next section will focus on issues that are specific to PKC’s role in pancreatic function.
2. PKC in the Pancreas
PKC has been shown to be involved in regulation of pancreatic exocrine and endocrine secretion. Methods such as immunohistochemistry and immunoblot analysis have enabled the identification of PKC isoforms in cells of the rodent endocrine and exocrine pancreas (Table 2, below).
The following sections (2a-d) focus predominantly on PKC in acini, although PKC distribution and function in other pancreatic cell types including ductal, islet and stellate cells are discussed in section 2e.
2a. PKC in the acinar cell
Cellular distribution
PKC isoforms α, δ, ε and ξ have been identified in rat pancreatic acini by immunoblot (47, 55). These isoforms were localized to the cytosol; addition of a phorbol ester, 12-O-tetradecanoylphorbol-13-acetate (TPA) causes translocation of these PKCs to membranes (4, 47). It should be noted that the results of studies of PKC isoform distribution in the exocrine pancreas have been inconsistent. For example, one study detected PKCε and -ξ in apical acini by immunofluorescence (IF) but not PKCα or -δ (4). Similarly, another investigation using IF did not find strong immunoreactivy for PKCα or -δ in pancreatic acini (28). Some of the differences among PKC studies may be due to the effectiveness of antisera used for immunoblot versus immunofluorescence. Translocation to membranes was observed for PKC isoforms α, δ, and ε, but not ξ in acini treated with physiological concentrations of the hormone, cholecystokinin (CCK-8; 100pM)(35). However, in another study, this CCK-8 stimulation resulted in translocation of PKCδ and -ε only (47).
2b. PKC and acinar cell secretion
PKC exhibits complex effects on acinar cell secretion. Several studies support a role for PKC in the intracellular control of acinar cell secretion. Secretagogues, such as cerulein, can stimulate calcium-independent amylase release through a PKC-dependent pathway (9). Phorbol esters such as 12-O-tetradecanoylphorbol-13-acetate (TPA) and synthetic diacylglycerol are known to stimulate modest amylase release alone and to enhance Ca2+ stimulated amylase release (8, 36, 60, 79). In permeabilized acini, phorbol esters (PMA, TPA) have been shown to enhance Ca2+ stimulated (29, 30, 44) as well as cAMP-dependent amylase release (29). However, prolonged pretreatment of acini with phorbol ester down regulates PKC activity and reduces CCK and carbachol stimulated amylase secretion (65). More recently, PKC inhibitors, such as bisindoylmalemide and its derivatives (i.e. GF109203X), have been shown to partially inhibit CCK and carbachol-induced amylase secretion (35). Various methods have also been used to study the effects of PKC isoforms on stimulated amylase release with physiological concentrations of CCK (35). In one study using pharmacologic inhibitors of PKC (GF109203X and rottlerin), CCK-induced (300pM) amylase release was reduced by ~30% or more. The study also found that Gö6976, a specific inhibitor of classical PKC (i.e. PKCα), did not inhibit amylase release (35) Thus, the reduction in amylase release was attributed to inhibition of PKCδ by rottlerin (35). Rottlerin, once thought to be a specific inhibitor of PKC has since been found to inhibit other PKC isoforms and other protein kinases (16, 33, 66, 68, 82). Adenoviral mediated over-expression of PKC isoforms demonstrated that over expression of PKCδ and -ε, but not -α, enhanced amylase release (35). In another study, using an isoform-specific PKCδ translocation inhibitor as well as PKCδ-/- mice, it was shown that there was no effect on stimulated amylase release over a range of CCK concentrations (0.001-100nM). Similarly, there was no difference in stimulated amylase release using carbachol (0.01-100 uM) as compared to wild-type (PKCδ +/+) (71). Thus, the reduction in amylase secretion previously attributed to inhibition of PKCδ in the first study may have been the result of inhibition of another PKC isoform (α, ε, ζ) or other protein kinases.
2c. PKC and acute pancreatitis
Acute pancreatitis is the result of numerous pathological events within the exocrine pancreas (pancreatic acinar cells), including inhibition of apical secretion, basolateral secretion, retention and premature activation of proteases, and elaboration of inflammatory mediators. Several PKC isoforms, including the conventional PKCα, novel PKCδ and -ε and atypical PKCξ have been shown to mediate some of these pancreatitis responses (13, 55, 56).
PKC activity and cellular distribution under pathological conditions
Stimulation of pancreatic acinar cells with supraphysiologic concentrations of CCK (10-100nM) have been shown to stimulate a rapid (2 min) rise in PKCδ and -ε activity and cause their translocation to membranes (47, 55). Furthermore, supraphysiologic CCK activated the PKCξ isoform, but did not initiate its translocation, while PKCα was neither activated nor translocated. In a separate study, however, supraphysiologic CCK caused PKCα activation and translocation (13). Additional studies have shown that ethanol alone can activate PKCε, while a combination of physiological CCK and ethanol activates PKCδ, -ε and -α, similar to supraphysiological CCK alone (56). Thus the sensitizing effects of ethanol may be mediated through the selective recruitment of specific PKC isoforms.
In pancreatic acinar cells, PKCα, -δ and -ε have a mainly apical, vesicular distribution (51). With ethanol and physiologic CCK treatment, PKCε translocates to perinuclear regions and the plasma membrane (56). This redistribution pattern was also seen in cells treated with the PKCε translocation activator. However, a different redistribution pattern was seen for PKCδ. While ethanol alone did not stimulate translocation, a combination of ethanol and physiological CCK did (56). PKC translocation has also been observed with supraphysiologic concentrations of cerulein (100nM). PKCε has been shown to translocate from an apical distribution to a supranuclear region upon supraphysiologic cerulein stimulation (Figure 4). It has also been shown to co-localize with GRAMP-92, an endosomal/lysosomal marker, in an area (supranuclear) where premature activation of digestive zymogens is believed to occur (70).
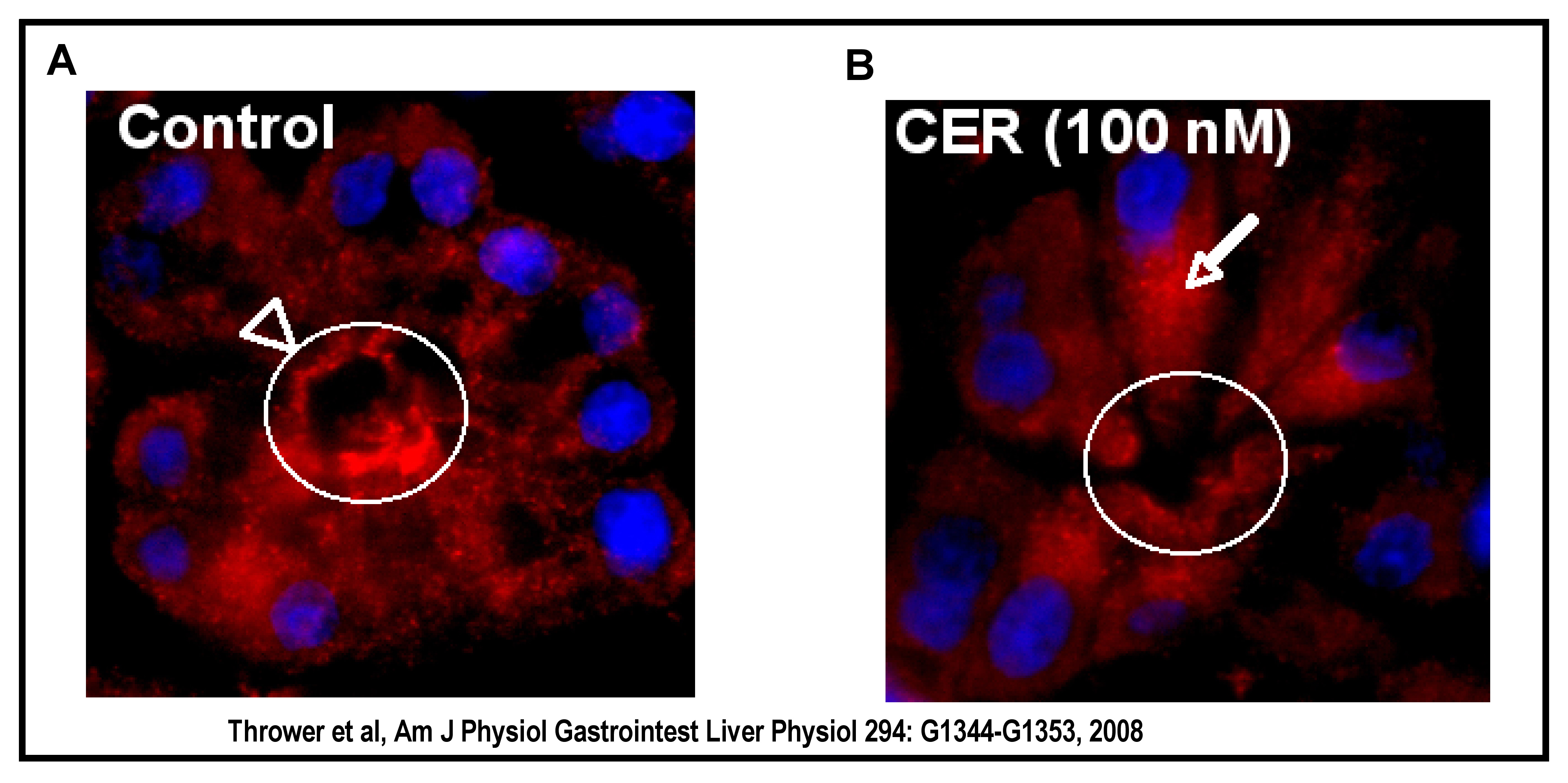
Figure 4. PKCε Translocation. In vivo, supraphysiological cerulein stimulates PKCε redistribution from an apical region to a supranuclear region of the acinar cell (apical region, arrowhead and circle; supranuclear region, arrow). PKCε (red); Nuclei are DAPI stained (blue).
Basolateral Secretion
In CCK-induced acute pancreatitis, decreased secretion into the pancreatic duct has been observed. Prior studies have indicated that a redirection of secretion from the apical membrane to the basolateral membrane may be a factor (18, 59). Ethanol (20mM) has been shown to inhibit physiological CCK-induced amylase secretion by blocking apical exocytosis with subsequent redirection of exocytosis to the basolateral membrane (13). Munc18c is a protein associated with the basolateral membrane of acinar cells where it binds and inhibits the SNARE protein, syntaxin-4 (18), thereby preventing basolateral exocytosis. PKCα, however, has been shown to phosphorylate Munc18c resulting in its dissociation from syntaxin-4. This leads to syntaxin-4 activation and SNARE complex formation, thus allowing basolateral exocytosis of zymogen granules (13, 18). Thus, under pathological conditions, apical secretion is disrupted and replaced with the less-efficient process of basolateral secretion.
Regulation of zymogen activation by PKC
The effects of PKC isoforms on premature zymogen activation have been studied in (i) a model of cerulein-induced pancreatitis with isolated rat acini and (ii) a reconstitution preparation with isolated pancreatic organelles and pancreatic cytosol. These studies were conducted with highly selective cell-permeable inhibitors of specific PKC isoforms (70).
In isolated pancreatic acini, activation of PKC with the phorbol ester 12-O-tetradecanoylphorbol-13-acetate (TPA; 200nM) had no affect on protease activity alone, but sensitized the acinar cell to cerulein (100 nM), resulting in enhanced trypsinogen and chymotrypsinogen activation. Pretreatment with either the broad spectrum PKC inhibitor GF109203X (Figure 5) or isoform specific inhibitors for PKCδ and -ε followed by cerulein addition resulted in a significant reduction in zymogen activation. In a reconstitution system, containing isolated pancreatic organelles and cytosol, with cofactors to generate active proteases, it was shown that effects of PKC were downstream of the CCK receptor (70).

Figure 5. Broad-spectrum PKC inhibitor GF-109203X reduces cerulein-induced (100nM) zymogen activation. The contribution of PKCδ in secretagogue-induced zymogen activation was verified in a later study using a genetic approach. Acini isolated from PKCδ -/- mice treated with a supraphysiologic concentration (100 nM) of the hormone cholecystokinin (CCK) showed much less zymogen activation than that seen in acini from wild type mice. Furthermore, other pancreatitis responses, including NF-κB activation, were also reduced by PKCδ deletion (70).
NF-κB activation
In acinar cells, supraphysiologic CCK, or its orthologue cerulein, activated NF-κB, an inflammatory mediator, through a PKC-dependent mechanism (20, 48, 67). Further, the broad-spectrum PKC inhibitor GF109203X inhibited NF-κB activity in a dose-dependent manner (48, 67). TNF-α has also been found to activate PKCδ, -e, and -ζ but not PKCα; however, only inhibition of -δ and -ε isoforms prevent NF-κB activation (55). The addition of ethanol has also been shown to sensitize acinar cells to this CCK effect. PKC isoform specific inhibitors blocked the effect of CCK with or without ethanol co-treatment. NF-κB activation was shown to be dependent on PKCδ and -ε activation through the use of isoform specific translocation activators (56). In a mouse model of cerulein-induced pancreatitis, PKCδ was also shown to activate NF-κB and mitogen activated protein kinases (MAPK) (49). Further, inhibition of PKC in this mouse model of acute pancreatitis showed a significant reduction in neutrophil and MCP-1 chemokine infiltration in the pancreas. Thus PKCδ appears to mediate pro-inflammatory responses in acute pancreatitis (49).
2d. Other targets of PKC
PKC substrates are phosphorylated at serine/threonine residues within a basic consensus sequence (R-X-X-S/T-X-R-X) (41). The pseudosubstrate domain of PKC maintains the kinases’ inactivity by mimicking the amino acids of a basic consensus sequence but with critical substitutions at the serine/threonine phosphorylation sites (39, 45). The list of downstream targets for PKC is extensive. A number of these targets are discussed below, in the context of the pancreas, and their relevance to pancreatic function and pathology.
Substance P and neurokinin-1 receptor
A recent study indicates that substance P, a neuropeptide, and neurokinin-1 receptor involvement in acute pancreatitis may be mediated by PKC. It was shown that cerulein induced substance P/neurokinin-1 receptor up-regulation is blocked by PKC inhibition. In particular, pretreatment with inhibitors of PKCα (Gö6976) and PKCδ (rottlerin, 1-10 μM) were responsible for this effect (32).
Binding proteins (MARCKS protein)
Various binding proteins, including the MARCKS protein, function as PKC substrates. These binding proteins are involved in intracellular or plasma membrane interactions with cytoskeletal elements (23). The MARCKS (myristoylated alanine-rich C kinase substrate) protein is involved in a host of activities such as the regulation of cellular migration and adhesion and endo-, exo- and phago-cytosis (2). This family of proteins is also believed to mediate regulation of the actin cytoskeleton (2). Whether they participate in actin redistribution during acute pancreatitis has not been determined.
Protein kinase D (PKD)
Protein Kinase D1 (formerly known as PKC μ) is a serine/threonine kinase that is distinct in terms of structure and regulatory properties from the isoforms comprising the PKC family. Activation of PKC involves PKC-dependent phosphorylation of Ser-744 and Ser-748 in the PKD1 activation loop. (50, 74-76). PKD1 was shown to mediate NF-κB activation induced by supraphysiologic CCK-8 and carbachol and to be a downstream target of PKCδ and -ε. This study identified PKD1 as a possible early convergent point for PKCδ and -ε (80). Further, knockdown or overexpression of PKD1 resulted in decreased or increased NFκB activity, respectively (80). PKC-dependent PKD phosphorylation has also been shown to regulate NF-κB activation in other cell types (12, 37, 62-64)). Inhibition of PKD with the chemical inhibitor, CRT0066101, was found to reduce zymogen activation, amylase secretion, and NF-kB activation (72).
Additional cellular targets of PKC
The CCK1 receptor has indirectly been shown to be a PKC substrate, but the effects of phosphorylation are unknown. CCK stimulates mitogen-activated protein kinase (MAPK) activation, which is PKC-dependent (15). This activation also results in activation of ras. Thus, PKC is involved in both the short-term responses associated with serine/threonine phosphorylation as well the long-term cellular responses associated with tyrosine phosphorylation (e.g. ras). PKC has also been shown to phosphorylate intracellular Ca2+ release channels such as the inositol [1,4,5] trisphosphate receptor (IP3R) (17). Whether PKC-mediated phosphorylation of IP3R contributes to sustained elevations of intracellular Ca2+ during acute pancreatitis is unclear. Further, PKC is known to phosphorylate and activate the Na+/H+ exchanger, thus modulating cytoplasmic pH (25). Fluctuations in acinar cell pH can promote pancreatitis responses, although it has not been determined if these are PKC-mediated (5).
2e. PKC effects in other pancreatic cell types
Several studies have demonstrated that PKC may be involved in various functions in other pancreatic cells types (duct epithelial cells, stellate cells, and islet cells) aside from acinar cells.
Duct cells
In duct cells PKC activation enhances exocytosis in a Ca2+ -independent manner (31). Protease-activated receptor-2 (PAR-2) has been found to mediate PKC-stimulated exocytosis (27). PKC also mediates bicarbonate (HCO3-) secretion. Secretin-stimulated HCO3- secretion is inhibited by substance P (SP). The inhibitory effect of SP is mediated by PKC. Activation of PKC results in reduced basal HCO3- secretion and complete block of secretin-stimulated secretion, whereas PKC inhibition reverses the inhibitory effect of SP (22).
Pancreatic stellate cells (PSC)
Pancreatic stellate cells (PSC) are best known for their role in promoting pancreatic fibrosis, but may also release acetylcholine and signal to acinar cells. Multiple studies have demonstrated a role for PKC in PSC activation (1, 19, 43). PKC is involved in regulating ethanol-induced PSC activation. Ethanol can activate PKC and other intracellular signaling molecules of the MAPK pathway while inhibition of PKC blocks PSC activation (1). A link between high glucose concentrations and PSC activation has been identified and may be mediated via a PKC-p38 MAPK pathway (43).
Islet cells (ß cells)
In islet cells, pharmacological activation of both cPKCs and nPKCs is necessary to induce insulin secretion, but only when combined with a stimulus for raising intracellular Ca2+ (81). Furthermore, studies in which PKC was either inhibited or down-regulated demonstrated that PKC is partially required for insulin secretion induced by muscarinic receptor agonists (3, 46, 69).
Knockout mouse models have been used more recently to address roles of nPKCs and aPKCs in β-cell function. Deletion of aPKCι/λ results in decreased glucose-stimulated insulin secretion (GSIS) (21), although this seems to be related to β-cell differentiation rather than stimulus–secretion coupling. A partial requirement for PKCδ in GSIS was also suggested from knockout mouse studies (73), although its activation by glucose was not observed in prior reports (77). Moreover, a role in GSIS was not supported when kinase-dead PKCδ was overexpressed in isolated rat islets using adenovirus (10). Therefore, a role in secretion for this isoform does not seem likely. Genetic deletion of PKCε however, results in a normalization of glucose tolerance in fat-fed mice due to an enhancement of insulin availability rather than improved insulin-sensitivity. This finding was confirmed by comparing GSIS from islets isolated from wild-type and PKCε-knockout animals chronically exposed to elevated fatty acids in tissue culture (6). The secretory defects induced under these conditions were prevented by deletion of PKCε.
The aPKCs are strongly implicated as regulators of β-cell proliferation. PKCζ, through mTOR activation, has been shown to modify the expression pattern of β-cell cycle molecules leading to increased β-cell replication and mass with a concomitant enhancement in β-cell function (6). Thus, PKC isoforms have varied functions in pancreatic ß-cells, ranging from secretion through to proliferation and apoptosis.
2f. Summary
PKC isoforms -α, -δ, -ε, and -ξ have been identified in the exocrine pancreas. In particular, various studies support a role for the novel PKC isoforms δ and ε in pathologic zymogen activation while PKCα may play a role in basolateral secretion. The PKC isoform involved in apical secretion has yet to be conclusively identified. PKC isoforms also have varied functions in other pancreatic cell types. Future studies may reveal more about the role of PKC isoforms in diseases such as pancreatitis and diabetes, and may have therapeutic implications for the disease.
3. Tools for the study of PKC
a. Activators
Phorbol ester 12-O-tetradecanoylphorbol-13-acetate (TPA); Sigma-Aldrich
Phorbol ester Phorbol-12,13-dibutyrate (PDB); LC Laboratories: Less potent than TPA but also less hydrophobic and thus easier to wash out of cells; Also tritium labeled form available.
Also please see:
Nelson and Alkon 2009 (38)
b. Inhibitors
In general, PKC inhibitors are classified in terms of their inhibition sites: 1) catalytic functional sites (ATP competitive binding), 2) effector sites (regulatory C1 domain binding), and 3) protein substrate sites. The putative PKC inhibitor staurosporine inhibits at the ATP binding site similar to the structurally derived bis-indolymalemides (i.e. GF-109203X, Gö6976, Gö6983). However, staurosporine is not selective for PKC thus the bis-indoylmalemide inhibitors were developed and even more specific inhibitors ruboxistaurin (LY333531) and enzastaurin (LY317615) have been designed (61).
PKC
- GF-109203X (Bisindolymaleimide I); Calbiochem, LC Laboratories. Inhibits most PKC isoforms
- Gö6976; Calbiochem, LC Laboratories. Inhibits conventional PKC isoforms (α, β, γ)
- Gö6983; Calbiochem, Sigma-Aldrich. A broad spectrum PKC inhibitor.
- Rottlerin; Calbiochem. Reported to be PKCδ specific but has nonspecific actions
- Chelerythrine, a selective cell-permeable plant derived benzophenanthridine alkaloid inhibitor; Sigma-Aldrich.
- Calphostin C, highly selective PKC inhibitor but requires light for activation;
Isoform specific translocation inhibitors originally developed by D. Mochly-Rosen (14) as reported for use in the pancreas by J. Reeve Jr. (University of California, Los Angeles) (55, 56)). The inhibitors were synthesized as an amino terminal extension to Drosophila antennapedia (R-QI-K-I-W-F-Q-N-R-R-M-K-W-K-K) for cell permeability.
- PKCδ translocation inhibitor (δV1-1: S-F-N-S-Y-E-L-G-S-L)
- PKCε translocation inhibitor (εV1-2: E-A-V-S-L-K-P-T)
- Scrambled peptide (L-S-E-T-K-P-A-V)
PKD:
1.CRT0066101; obtained from Cancer Research Technology, London, UK
c. Antibodies
PKC:
- Rabbit anti-PKCδ or -ε, 1:200 (WB), 1:100 (IF); Santa Cruz Biotechnology
- Rabbit anti-PKCα; Cell Signaling
PKD:
- PKD1/PKD2 C-20; Santa Cruz
- Phosphoserine 744/748 PKD/PKD1 (detects primarily the phosphorylated state of Ser-744; an indirect marker of activation); Cell Signaling
- Phosphoserine 916 PKD/PKD1, indirect marker of activation; Cell Signaling
d. Viral Vectors
1. NF-kappaB activation: Adenoviral transfer of active subunit (RelA/p65 or Adp65) as described by Chen, Logsdon and colleagues (11)
2. Wild type and dominant negative adenoviral vectors (PKC-α, -δ, -ε) as described by Braz et al (7).
e. Mouse lines
PKCα (-/-) mice as described by Letiges et al (34).
PKCδ (-/-) mice and littermate wild-type (+/+; C57BL/6 background) mice. Bred at Veterans Affairs Greater Los Angeles Health Care System, Los Angeles, California. Mice initially generated by Miyamoto et al [2002] by replacement of the first and second exons of PKC genes with a neomycin-resistance cassette.
PKCε (-/-) mice and wild type (+/+; C57BL/6 background) mice. Generated by intercrossing 129SvJaex C57Bl/6 hybrid PKCε+/- (24, 26).
4.References
- Apte MV, Pirola RC, and Wilson JS. Battle-scarred pancreas: role of alcohol and pancreatic stellate cells in pancreatic fibrosis. J Gastroenterol Hepatol 21 Suppl 3: S97-S101, 2006. PMID 16958684
- Arbuzova A, Schmitz AA, and Vergeres G. Cross-talk unfolded: MARCKS proteins. Biochem J 362: 1-12, 2002. PMID 11829734
- Arkhammar P, Nilsson T, Welsh M, Welsh N, and Berggren PO. Effects of protein kinase C activation on the regulation of the stimulus-secretion coupling in pancreatic beta-cells. Biochem J 264: 207-215, 1989. PMID 2690820
- Bastani B, Yang L, Baldassare JJ, Pollo DA, and Gardner JD. Cellular distribution of isoforms of protein kinase C (PKC) in pancreatic acini. Biochim Biophys Acta 1269: 307-315, 1995. PMID 7495885
- Bhoomagoud M, Jung T, Atladottir J, Kolodecik TR, Shugrue C, Chaudhuri A, Thrower EC, and Gorelick FS. Reducing extracellular pH sensitizes the acinar cell to secretagogue-induced pancreatitis responses in rats. Gastroenterology 137: 1083-1092, 2009. PMID 19454288
- Biden TJ, Schmitz-Peiffer C, Burchfield JG, Gurisik E, Cantley J, Mitchell CJ, and Carpenter L. The diverse roles of protein kinase C in pancreatic beta-cell function. Biochem Soc Trans 36: 916-919, 2008. PMID 18793161
- Braz JC, Bueno OF, De Windt LJ, and Molkentin JD. PKC alpha regulates the hypertrophic growth of cardiomyocytes through extracellular signal-regulated kinase1/2 (ERK1/2). J Cell Biol 156: 905-919, 2002. PMID 11864961
- Bruzzone R. The molecular basis of enzyme secretion. Gastroenterology 99: 1157-1176, 1990. PMID 2118462
- Bruzzone R, Regazzi R, and Wollheim CB. Caerulein causes translocation of protein kinase C in rat acini without increasing cytosolic free Ca2+. Am J Physiol 255: G33-39, 1988. PMID 2455450
- Carpenter L, Mitchell CJ, Xu ZZ, Poronnik P, Both GW, and Biden TJ. PKC alpha is activated but not required during glucose-induced insulin secretion from rat pancreatic islets. Diabetes 53: 53-60, 2004. PMID 14693697
- Chen X, Ji B, Han B, Ernst SA, Simeone D, and Logsdon CD. NF-kappaB activation in pancreas induces pancreatic and systemic inflammatory response. Gastroenterology 122: 448-457, 2002. PMID 11832459
- Chiu TT, Leung WY, Moyer MP, Strieter RM, and Rozengurt E. Protein kinase D2 mediates lysophosphatidic acid-induced interleukin 8 production in nontransformed human colonic epithelial cells through NF-kappaB. Am J Physiol Cell Physiol 292: C767-777, 2007. PMID 16928771
- Cosen-Binker LI, Lam PP, Binker MG, Reeve J, Pandol S, and Gaisano HY. Alcohol/cholecystokinin-evoked pancreatic acinar basolateral exocytosis is mediated by protein kinase C alpha phosphorylation of Munc18c. J Biol Chem 282: 13047-13058, 2007. PMID 17324928
- Csukai M, and Mochly-Rosen D. Pharmacologic modulation of protein kinase C isozymes: the role of RACKs and subcellular localisation. Pharmacol Res 39: 253-259, 1999. PMID 10208754
- Dabrowski A, VanderKuur JA, Carter-Su C, and Williams JA. Cholecystokinin stimulates formation of shc-grb2 complex in rat pancreatic acinar cells through a protein kinase C-dependent mechanism. J Biol Chem 271: 27125-27129, 1996. PMID 8900204
- Davies SP, Reddy H, Caivano M, and Cohen P. Specificity and mechanism of action of some commonly used protein kinase inhibitors. Biochem J 351: 95-105, 2000. PMID 10998351
- Ferris CD, Huganir RL, Bredt DS, Cameron AM, and Snyder SH. Inositol trisphosphate receptor: phosphorylation by protein kinase C and calcium calmodulin-dependent protein kinases in reconstituted lipid vesicles. Proc Natl Acad Sci U S A 88: 2232-2235, 1991. PMID 1848697
- Gaisano HY, Lutz MP, Leser J, Sheu L, Lynch G, Tang L, Tamori Y, Trimble WS, and Salapatek AM. Supramaximal cholecystokinin displaces Munc18c from the pancreatic acinar basal surface, redirecting apical exocytosis to the basal membrane. J Clin Invest 108: 1597-1611, 2001. PMID 11733555
- Hama K, Ohnishi H, Aoki H, Kita H, Yamamoto H, Osawa H, Sato K, Tamada K, Mashima H, Yasuda H, and Sugano K. Angiotensin II promotes the proliferation of activated pancreatic stellate cells by Smad7 induction through a protein kinase C pathway. Biochem Biophys Res Commun 340: 742-750, 2006. PMID 16380081
- Han B, and Logsdon CD. CCK stimulates mob-1 expression and NF-kappaB activation via protein kinase C and intracellular Ca(2+). Am J Physiol Cell Physiol 278: C344-351, 2000. PMID 10666030
- Hashimoto N, Kido Y, Uchida T, Matsuda T, Suzuki K, Inoue H, Matsumoto M, Ogawa W, Maeda S, Fujihara H, Ueta Y, Uchiyama Y, Akimoto K, Ohno S, Noda T, and Kasuga M. PKClambda regulates glucose-induced insulin secretion through modulation of gene expression in pancreatic beta cells. J Clin Invest 115: 138-145, 2005. PMID 15630453
- Hegyi P, Rakonczay Z, Jr., Tiszlavicz L, Varro A, Toth A, Racz G, Varga G, Gray MA, and Argent BE. Protein kinase C mediates the inhibitory effect of substance P on HCO3- secretion from guinea pig pancreatic ducts. Am J Physiol Cell Physiol 288: C1030-1041, 2005. PMID 15625303
- Jaken S. Protein kinase C isozymes and substrates. Curr Opin Cell Biol 8: 168-173, 1996. PMID 8791416
- Kaiser JP, Beier JI, Zhang J, David Hoetker J, von Montfort C, Guo L, Zheng Y, Monia BP, Bhatnagar A, and Arteel GE. PKCepsilon plays a causal role in acute ethanol-induced steatosis. Arch Biochem Biophys 482: 104-111, 2009. PMID 19022218
- Khalil R. Regulation of Vascular Smooth Muscle Function: Chapter 6, Protein Kinase C. San Rafael (CA): Morgan & Claypool Life Sciences, 2010.
- Khasar SG, Lin YH, Martin A, Dadgar J, McMahon T, Wang D, Hundle B, Aley KO, Isenberg W, McCarter G, Green PG, Hodge CW, Levine JD, and Messing RO. A novel nociceptor signaling pathway revealed in protein kinase C epsilon mutant mice. Neuron 24: 253-260, 1999. PMID 10677042
- Kim MH, Choi BH, Jung SR, Sernka TJ, Kim S, Kim KT, Hille B, Nguyen TD, and Koh DS. Protease-activated receptor-2 increases exocytosis via multiple signal transduction pathways in pancreatic duct epithelial cells. J Biol Chem 283: 18711-18720, 2008. PMID 18448425
- Kim MJ, Lee YS, Lee KH, Min DS, Yoon SH, Hahn SJ, Kim MS, and Jo YH. Site-specific localization of protein kinase C isoforms in rat pancreas. Pancreatology 1: 36-42, 2001. PMID 12120266
- Kimura T, Imamura K, Eckhardt L, and Schulz I. Ca2+-, phorbol ester-, and cAMP-stimulated enzyme secretion from permeabilized rat pancreatic acini. Am J Physiol 250: G698-708, 1986. PMID 2422955
- Kitagawa M, Williams JA, and De Lisle RC. Amylase release from streptolysin O-permeabilized pancreatic acini. Am J Physiol 259: G157-164, 1990. PMID 1696432
- Koh DS, Moody MW, Nguyen TD, and Hille B. Regulation of exocytosis by protein kinases and Ca(2+) in pancreatic duct epithelial cells. J Gen Physiol 116: 507-520, 2000. PMID 11004201
- Koh YH, Tamizhselvi R, Moochhala S, Bian JS, and Bhatia M. Role of protein kinase C in caerulein induced expression of substance P and neurokinin-1-receptors in murine pancreatic acinar cells. J Cell Mol Med 15: 2139-2149, 2011. PMID 20973912
- Leitges M, Elis W, Gimborn K, and Huber M. Rottlerin-independent attenuation of pervanadate-induced tyrosine phosphorylation events by protein kinase C-delta in hemopoietic cells. Lab Invest 81: 1087-1095, 2001. PMID 11502860
- Leitges M, Plomann M, Standaert ML, Bandyopadhyay G, Sajan MP, Kanoh Y, and Farese RV. Knockout of PKC alpha enhances insulin signaling through PI3K. Mol Endocrinol 16: 847-858, 2002. PMID 11923480
- Li C, Chen X, and Williams JA. Regulation of CCK-induced amylase release by PKC-delta in rat pancreatic acinar cells. Am J Physiol Gastrointest Liver Physiol 287: G764-771, 2004. PMID 15217780
- Merritt JE, and Rubin RP. Pancreatic amylase secretion and cytoplasmic free calcium. Effects of ionomycin, phorbol dibutyrate and diacylglycerols alone and in combination. Biochem J 230: 151-159, 1985. PMID 2413839
- Mihailovic T, Marx M, Auer A, Van Lint J, Schmid M, Weber C, and Seufferlein T. Protein kinase D2 mediates activation of nuclear factor kappaB by Bcr-Abl in Bcr-Abl+ human myeloid leukemia cells. Cancer Res 64: 8939-8944, 2004. PMID 15604256
- Nelson TJ, and Alkon DL. Neuroprotective versus tumorigenic protein kinase C activators. Trends Biochem Sci 34: 136-145, 2009. PMID 19233655
- Newton AC. Protein kinase C: poised to signal. Am J Physiol Endocrinol Metab 298: E395-402, 2010. PMID 19934406
- Nguyen BT, and Dessauer CW. Relaxin stimulates protein kinase C zeta translocation: requirement for cyclic adenosine 3',5'-monophosphate production. Mol Endocrinol 19: 1012-1023, 2005. PMID 15604116
- Nishikawa K, Toker A, Johannes FJ, Songyang Z, and Cantley LC. Determination of the specific substrate sequence motifs of protein kinase C isozymes. J Biol Chem 272: 952-960, 1997. PMID 8995387
- Nishizuka Y. The protein kinase C family and lipid mediators for transmembrane signaling and cell regulation. Alcohol Clin Exp Res 25: 3S-7S, 2001. PMID 11391043
- Nomiyama Y, Tashiro M, Yamaguchi T, Watanabe S, Taguchi M, Asaumi H, Nakamura H, and Otsuki M. High glucose activates rat pancreatic stellate cells through protein kinase C and p38 mitogen-activated protein kinase pathway. Pancreas 34: 364-372, 2007. PMID 17414061
- O'Sullivan AJ, and Jamieson JD. Activation of protein kinase C is not an absolute requirement for amylase release from permeabilized rat pancreatic acini. Biochem J 285 ( Pt 2): 597-601, 1992. PMID 1379047
- Pearce LR, Komander D, and Alessi DR. The nuts and bolts of AGC protein kinases. Nat Rev Mol Cell Biol 11: 9-22, 2010. PMID 20027184
- Persaud SJ, Jones PM, Sugden D, and Howell SL. The role of protein kinase C in cholinergic stimulation of insulin secretion from rat islets of Langerhans. Biochem J 264: 753-758, 1989. PMID 2695065
- Pollo DA, Baldassare JJ, Honda T, Henderson PA, Talkad VD, and Gardner JD. Effects of cholecystokinin (CCK) and other secretagogues on isoforms of protein kinase C (PKC) in pancreatic acini. Biochim Biophys Acta 1224: 127-138, 1994. PMID 7524684
- Rakonczay Z, Jr., Hegyi P, Takacs T, McCarroll J, and Saluja AK. The role of NF-kappaB activation in the pathogenesis of acute pancreatitis. Gut 57: 259-267, 2008. PMID 17675325
- Ramnath RD, Sun J, and Bhatia M. PKC delta mediates pro-inflammatory responses in a mouse model of caerulein-induced acute pancreatitis. J Mol Med (Berl) 88: 1055-1063, 2010. PMID 20582580
- Rey O, Reeve JR, Jr., Zhukova E, Sinnett-Smith J, and Rozengurt E. G protein-coupled receptor-mediated phosphorylation of the activation loop of protein kinase D: dependence on plasma membrane translocation and protein kinase Cepsilon. J Biol Chem 279: 34361-34372, 2004. PMID 15190080
- Rodriguez-Martin E, Boyano-Adanez MC, Bodega G, Martin M, Hernandez C, Quin Y, Vadillo M, and Arilla-Ferreiro E. Redistribution of protein kinase C isoforms in rat pancreatic acini during lactation and weaning. FEBS Lett 445: 356-360, 1999. PMID 10094489
- Ron D, Luo J, and Mochly-Rosen D. C2 region-derived peptides inhibit translocation and function of beta protein kinase C in vivo. J Biol Chem 270: 24180-24187, 1995. PMID 7592622
- Ron D, and Mochly-Rosen D. An autoregulatory region in protein kinase C: the pseudoanchoring site. Proc Natl Acad Sci U S A 92: 492-496, 1995. PMID 7831317
- Rosse C, Linch M, Kermorgant S, Cameron AJ, Boeckeler K, and Parker PJ. PKC and the control of localized signal dynamics. Nat Rev Mol Cell Biol 11: 103-112, 2010. PMID 20094051
- Satoh A, Gukovskaya AS, Nieto JM, Cheng JH, Gukovsky I, Reeve JR, Jr., Shimosegawa T, and Pandol SJ. PKC-delta and -epsilon regulate NF-kappaB activation induced by cholecystokinin and TNF-alpha in pancreatic acinar cells. Am J Physiol Gastrointest Liver Physiol 287: G582-591, 2004. PMID 15117677
- Satoh A, Gukovskaya AS, Reeve JR, Jr., Shimosegawa T, and Pandol SJ. Ethanol sensitizes NF-kappaB activation in pancreatic acinar cells through effects on protein kinase C-epsilon. Am J Physiol Gastrointest Liver Physiol 291: G432-438, 2006. PMID 16574982
- Schaefer M, Albrecht N, Hofmann T, Gudermann T, and Schultz G. Diffusion-limited translocation mechanism of protein kinase C isotypes. Faseb J 15: 1634-1636, 2001. PMID 11427510
- Schechtman D, and Mochly-Rosen D. Adaptor proteins in protein kinase C-mediated signal transduction. Oncogene 20: 6339-6347, 2001. PMID 11607837
- Scheele G, Adler G, and Kern H. Exocytosis occurs at the lateral plasma membrane of the pancreatic acinar cell during supramaximal secretagogue stimulation. Gastroenterology 92: 345-353, 1987. PMID 3792771
- Singh J. Phorbol ester (TPA) potentiates noradrenaline and acetylcholine-evoked amylase secretion in the rat pancreas. FEBS Lett 180: 191-195, 1985. PMID 2578414
- Son YK, Hong da H, Kim DJ, Firth AL, and Park WS. Direct effect of protein kinase C inhibitors on cardiovascular ion channels. BMB Rep 44: 559-565, 2011. PMID 21944247
- Storz P, Doppler H, and Toker A. Activation loop phosphorylation controls protein kinase D-dependent activation of nuclear factor kappaB. Mol Pharmacol 66: 870-879, 2004. PMID 15226414
- Storz P, Doppler H, and Toker A. Protein kinase Cdelta selectively regulates protein kinase D-dependent activation of NF-kappaB in oxidative stress signaling. Mol Cell Biol 24: 2614-2626, 2004. PMID 15024053
- Storz P, and Toker A. Protein kinase D mediates a stress-induced NF-kappaB activation and survival pathway. Embo J 22: 109-120, 2003. PMID 12505989
- Sung CK, Hootman SR, Stuenkel EL, Kuroiwa C, and Williams JA. Downregulation of protein kinase C in guinea pig pancreatic acini: effects on secretion. Am J Physiol 254: G242-248, 1988. PMID 2450470
- Susarla BT, and Robinson MB. Rottlerin, an inhibitor of protein kinase Cdelta (PKCdelta), inhibits astrocytic glutamate transport activity and reduces GLAST immunoreactivity by a mechanism that appears to be PKCdelta-independent. J Neurochem 86: 635-645, 2003. PMID 12859677
- Tando Y, Algul H, Wagner M, Weidenbach H, Adler G, and Schmid RM. Caerulein-induced NF-kappaB/Rel activation requires both Ca2+ and protein kinase C as messengers. Am J Physiol 277: G678-686, 1999. PMID 10484394
- Tapia JA, Jensen RT, and Garcia-Marin LJ. Rottlerin inhibits stimulated enzymatic secretion and several intracellular signaling transduction pathways in pancreatic acinar cells by a non-PKC-delta-dependent mechanism. Biochim Biophys Acta 1763: 25-38, 2006. PMID 16364465
- Thams P, Capito K, Hedeskov CJ, and Kofod H. Phorbol-ester-induced down-regulation of protein kinase C in mouse pancreatic islets. Potentiation of phase 1 and inhibition of phase 2 of glucose-induced insulin secretion. Biochem J 265: 777-787, 1990. PMID 2407236
- Thrower EC, Osgood S, Shugrue CA, Kolodecik TR, Chaudhuri AM, Reeve JR, Jr., Pandol SJ, and Gorelick FS. The novel protein kinase C isoforms -delta and -epsilon modulate caerulein-induced zymogen activation in pancreatic acinar cells. Am J Physiol Gastrointest Liver Physiol 294: G1344-1353, 2008. PMID 18388183
- Thrower EC, Wang J, Cheriyan S, Lugea A, Kolodecik TR, Yuan J, Reeve JR, Jr., Gorelick FS, and Pandol SJ. Protein kinase C delta-mediated processes in cholecystokinin-8-stimulated pancreatic acini. Pancreas 38: 930-935, 2009. PMID 19752773
- Thrower EC, Yuan J, Usmani A, Liu Y, Jones C, Minervini SN, Alexandre M, Pandol SJ, and Guha S. A novel protein kinase D inhibitor attenuates early events of experimental pancreatitis in isolated rat acini. Am J Physiol Gastrointest Liver Physiol 300: G120-129, 2011. PMID 20947701
- Uchida T, Iwashita N, Ohara-Imaizumi M, Ogihara T, Nagai S, Choi JB, Tamura Y, Tada N, Kawamori R, Nakayama KI, Nagamatsu S, and Watada H. Protein kinase Cdelta plays a non-redundant role in insulin secretion in pancreatic beta cells. J Biol Chem 282: 2707-2716, 2007. PMID 17135234
- Valverde AM, Sinnett-Smith J, Van Lint J, and Rozengurt E. Molecular cloning and characterization of protein kinase D: a target for diacylglycerol and phorbol esters with a distinctive catalytic domain. Proc Natl Acad Sci U S A 91: 8572-8576, 1994. PMID 8078925
- Waldron RT, Iglesias T, and Rozengurt E. The pleckstrin homology domain of protein kinase D interacts preferentially with the eta isoform of protein kinase C. J Biol Chem 274: 9224-9230, 1999. PMID 10092595
- Waldron RT, Rey O, Iglesias T, Tugal T, Cantrell D, and Rozengurt E. Activation loop Ser744 and Ser748 in protein kinase D are transphosphorylated in vivo. J Biol Chem 276: 32606-32615, 2001. PMID 11410586
- Warwar N, Efendic S, Ostenson CG, Haber EP, Cerasi E, and Nesher R. Dynamics of glucose-induced localization of PKC isoenzymes in pancreatic beta-cells: diabetes-related changes in the GK rat. Diabetes 55: 590-599, 2006. PMID 16505220
- Webb BL, Hirst SJ, and Giembycz MA. Protein kinase C isoenzymes: a review of their structure, regulation and role in regulating airways smooth muscle tone and mitogenesis. Br J Pharmacol 130: 1433-1452, 2000. PMID 10928943
- Williams JA, Burnham DB, and Hootman SR. Cellular Regulation of Pancreatic Secretion. . In: Comprehensive PhysiologyJohn Wiley & Sons, Inc., 2011, p. 419-441.
- Yuan J, Lugea A, Zheng L, Gukovsky I, Edderkaoui M, Rozengurt E, and Pandol SJ. Protein kinase D1 mediates NF-kappaB activation induced by cholecystokinin and cholinergic signaling in pancreatic acinar cells. Am J Physiol Gastrointest Liver Physiol 295: G1190-1201, 2008. PMID 18845574
- Zawalich W, Brown C, and Rasmussen H. Insulin secretion: combined effects of phorbol ester and A23187. Biochem Biophys Res Commun 117: 448-455, 1983. PMID 6229253
- Zhao H, Tian W, and Cohen DM. Rottlerin inhibits tonicity-dependent expression and action of TonEBP in a PKCdelta-independent fashion. Am J Physiol Renal Physiol 282: F710-717, 2002. PMID 11880333