Entry Version:
Citation:
Pancreapedia: Exocrine Pancreas Knowledge Base, DOI: 10.3998/panc.2012.4
Attachment | Size |
---|---|
![]() | 253.33 KB |
Gene Symbol: Epac1, Rapgef3, Epac2, Rapgef4
1.General Information
Epac (Exchange protein directly activated by cAMP) is an important effector for cAMP, which was originally thought to act only through protein kinase A (PKA) or cyclic nucleotide binding ion channels. The two variants of Epac, Epac1 and Epac2, were identified in 1998 by two studies. The first study was through data base screening for a molecule with RapGEF activity and homology to cyclic AMP binding proteins (8) and the second by a screen for cyclic AMP binding proteins in brain (23). Both Epac1 and Epac2 have GEF activity for Rap1 and were alternatively known as cAMPGEF1 and cAMPGEF2. Because calcium and diacylglycerol activated RapGEFs, known as CalDAG-GEFs which were discovered earlier, the official gene name for Epacs are Rapgef3 and Rapgef4. The Drosophila gene, however, is Epac and we will use this designation in the remainder of this review.
Epac Sequence and Structure
Epac1 mRNA is ubiquitously expressed, whereas Epac2 is predominantly expressed in brain and endocrine tissues (23). Epac1 and 2 are 881 and 993 amino acid homologous proteins with Epac2 having a N-terminal extension. They share common domain structures within a N-terminal regulatory region and a C-terminal catalytic domain as shown in Figure 1 (2,15,19). The N-terminal region possesses one (Epac1) or two (Epac2) CNB (Cyclic Nucleotide Binding) domains and a DEP (Disheveled, Egl-10, and Pleckstrin) domain. The C-terminal region contains a CDC25-homology domain, a REM (Ras Exchange Motif) and a RA (Ras Association) domain. The functional cAMP-binding site on Epac1 and Epac2 binds cAMP in vitro with similar affinity to that of PKA (Kd 2.8 μM for Epac1 and 1.2 μM for Epac2 compared with 2.9 μM for PKA). Epac2 has an additional low affinity cAMP-binding domain at its N-terminus (Kd 87 μM), which has an uncertain function since it is not required for cAMP-induced Rap1 activation (8). While in vitro cAMP affinity for Epac and PKA are virtually the same, the concentration of cAMP required for half-maximal activation of Epac1 is around 30 μM compared with ~1 μM for PKA. The difference in these sensitivities may be due to positive co-operativity between the two cAMP-binding sites on PKA, which is lacking on Epac1 which has only a single cAMP-binding site (7). This means that PKA can be activated by small increases in cAMP levels, but higher levels of cAMP are required to activate Epac-mediated signaling. The crystal structure of Epac2 has been obtained for both inactive and active configurations (32, 33). Upon cAMP binding, the regulatory region of the molecule moves to expose the catalytic region. The DEP domain is involved in membrane targeting of the active molecule and the REM domain is participates in Ras activation when the two molecules are closely approximated. For further details relating to the structural dynamics in the activation of Epac, see (16).
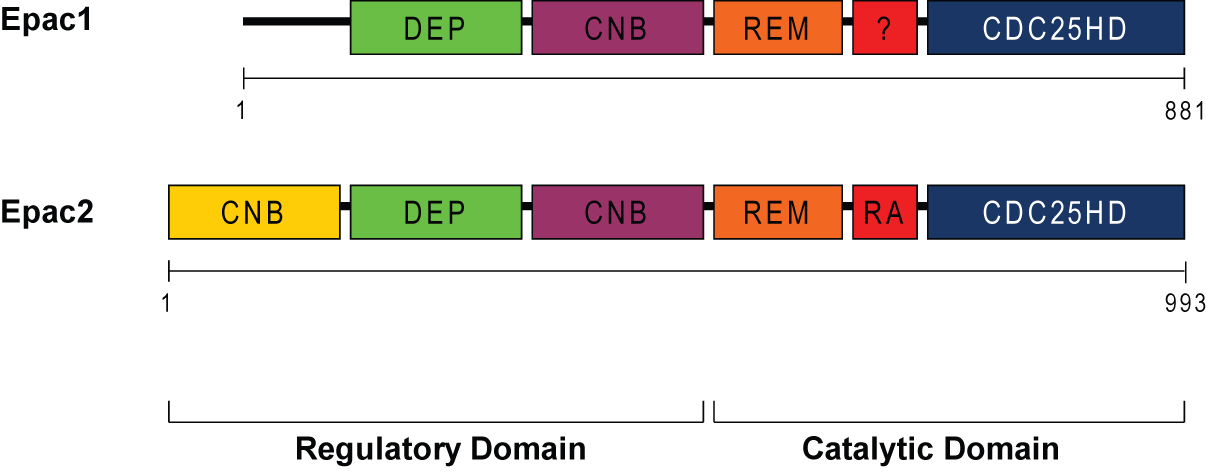
Figure 1: Domain structure of Epac1 and Epac2. Both Epac1 (881 amino acids) and Epac2 (993 amino acids) contain two domains: regulatory and catalytic. The regulatory region has the cyclic nucleotide-binding domain (CNB), the Desheveled-Egl-10-Pleckstrin (DEP), which is involved in membrane localization. The catalytic region has the CDC25-homology domain, which is responsible for the guanine-nucleotide exchange activity, and the Ras exchange motif (REM), which stabilizes the catalytic helix of CDC25HD, and the Ras-association (RA) domain, which is a protein interaction motif.
Epac Selective cAMP Analogs
An important advance in the ability to study Epac was the development of cAMP analogs that are selective for Epac or PKA (10). Selectivity for Epac is conferred by the substitution of an O-Methyl group on the ribose moiety of cAMP at the 2’ carbon position allowing the 2’-O-Methyl-cAMP derivative (Figure 2) to act as an Epac agonist. To enhance lipophilicity, this modification is usually combined with addition of a chloro-phenylthio moiety to yield 8-(4-chloro-phenylthio)-2’-O-methyladenosine-3’,5’-cyclic monophosphate (pCPT-2-O-Me-cAMP). Such analogs are sometimes referred to as ESCAs (Epac-selective cAMP analogs). Conversely, N6-benzoyl-cAMP (6-Bnz-cAMP) (Fig. 2) is an agonist for PKA, but inactive with regard to Epac. Evidence as to whether ESCAs are activating PKA can be by the simultaneous use of PKA inhibitors. Currently, no inhibitors of Epac proteins are available. The Arf-GEF inhibitor, brefeldin, was shown to be an inhibitor of Epac function, but is likely non-selective (15, 40).
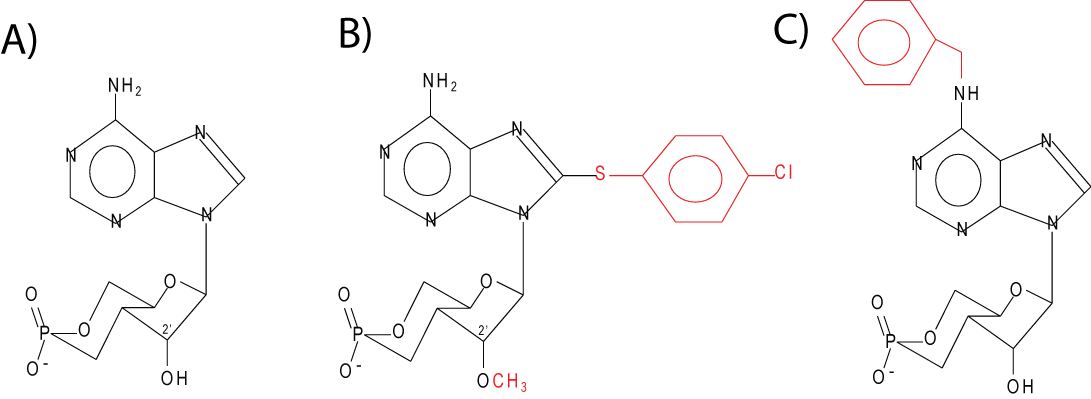
Figure 2. Molecular structure of cAMP (A) and analogs specific for Epac, 8-pCPT-2-O-Me-cAMP, (B) and PKA, 6-Bnz-cAMP (C).
Biological Functions of Epacs
The major function of Epac is to serve as the guanine nucleotide exchange or GEF function for Rap1 and Rap2. Epac and Rap play major roles in the regulation of cardiac function, secretion, vascular function, proliferation, and inflammation and study of these processes has revealed downstream targets (1). In the heart, Epac acts through Rap to mediate the action of β-adrenergic receptors to activate phospholipase Cε and mobilize intracellular Ca2+ (30). This leads to activation of PKC and CaMKII and enhanced phosphorylation of ryanodine receptors (29). Epac also plays a role in cardiac hypertrophy, but this action was reported to be independent of Rap, and to involve calcineurin and CaMKII (27, 28).
Epac regulates exocytosis in neurons, endocrine and exocrine cells. The best studied process is insulin secretion by islet beta cells. cAMP-induced by incretin receptors, such as those for GLP-1 and GIP, is known to potentiate glucose-stimulated insulin secretion. This is now known to be mediated by Epac2, the primary form of Epac in beta cells (21, 31). Epac mediates the cAMP dependent mobilization of Ca2+ from intracellular stores (18). This action, as in cardiac muscle, involves PKCε and is abolished in PKCε KO mice (9). In addition to potentiating Ca2+ signaling through an effect on ryanodine receptors, Epac may bind Rim2, an effector of the small G protein Rab3 and another protein, Piccolo and mediate recruitment of secretory granules to release sites on the plasma membrane in a process known as priming (11, 22, 24, 37). A number of reviews are available on this subject (19, 25, 35). Epac has been shown to play a role in neurosecretion in the giant synapse of the Calyx of Held, hippocampal neurons, and in the crayfish neuromuscular junction (14, 20, 44). Through such effects, Epac plays a role in learning and memory. Epac also contributes to neurite outgrowth, neuronal differentiation and axon regeneration (39). In this study of PACAP-mediated deuronal differentiation, Rap was not essential but rather another small G protein Rit that is related to Ras was involved. A role of Epac in exocrine secretion is considered in Section 2.
Epac plays a role in vascular function in the endothelial layer of blood vessels where cAMP strengthens the epithelial barrier and counteracts the effect of inflammatory mediators (6, 13). This involves both cadherin molecules linking to the underlying actin cytoskeleton and the formation of junctional complexes both of which are mediated by Rap1. In addition to the vascular endothelium, cAMP signaling also regulates inflammation through actions on leukocytes, most of which express Epac1.
cAMP is known to play a role in many cells in regulating cell proliferation through positive or negative effects on mitogenesis depending on the cellular context and Epac and/or Rap1 may be involved (1). For example, Epac acts synergistically with PKA to enhance mitogenesis mediated by cAMP in rat thyroid cells (17). Some of this proliferative effect of Epac may be mediated by Rap1 activating the ERK cascade in a Ras independent manner involving BRaf (12). Less is known as to the negative effects of cAMP on cell division, but overexpression of Epac or Rap1 inhibits proliferation of Jurkat T-cells (3). In addition to effects on mitogenesis, Epac and Rap1 may mediate some of the effects of cAMP on cell differentiation (1).
2. Epac in the Exocrine Pancreas
cAMP induced by secretin and VIP is known to regulate both acinar and ductal cell function. Effects of cAMP on acinar cells include potentiation of enzyme secretion and sensitization to intracellular digestive enzyme activation (5, 42). To evaluate possible effects of Epac as a mediator of cAMP, Chaudhuri et al utilized 8-pCPT-2’-O-Me-cAMP and showed that it enhanced both carbachol-induced amylase secretion and zymogen activation in rat acini and the effect was not blocked by PKI (4). By contrast, the Epac agonist had no effect on CREB phosphorylation. They concluded that cyclic AMP could act by both PKA and Epac pathways. In a study of Rap1 activation, Sabbatini et al showed that Epac1, but not Epac2, was present in mouse pancreatic exocrine cells (34). They found that the effect of forskolin to activate Rap1 were mimicked by 8Br-cAMP and 8-pCPT-2’-O-Me-cAMP and this activation was not blocked by a PKA inhibitor. Using a polyclonal antibody against Epac1 from Abcam, Epac1 was localized by immunohistochemistry to the granular pole of the acini where it overlapped Rap1 which is known to be on the ZG membrane (See Molecule Page for Rap1). Epac1 however has not been identified on the ZG. Thus the Epac/Rap1 pathway appeared to mediate cyclic AMP stimulated amylase secretion. At present the downstream mediators of Rap1 are unknown. In a third study in rat acini, the effect of cAMP to enhance the speed of Ca2+ waves was shown to be mediated in part by PKA and in part by Epac (36). This effect involves the ryanodine receptor and Ca2+ release. At present there is no evidence for a role for Epac in pancreatic duct cells and the effect of cAMP on ductal secretion is believed mediated by PKA phosphorylation of CFTR. However, we are not aware any study that has evaluated the effects of ESCA.
Epac1 has also been identified in the parotid gland (38). Unlike the pancreatic acinar cell, isoproterenol-induced amylase secretion from parotid acinar cells is largely mediated by cAMP. This secretion has been shown in part to involve Epac as well as the predominant PKA component (38, 43).
A published letter to the Editor suggests that Epac1 is overexpressed in human pancreatic adenocarcinoma and can mediate the inhibition of growth in response to cAMP seen in Panc1 and MiaPaCa cell lines (26). Further investigations on the role of Epac in neoplasia are warranted.
3. Tools to Study Epac
a. cDNA clones
cDNA clones are available from Origene and GeneCopeia. A dominant negative Epac1 has been described (17).
b. Antibodies
In our earlier work (34) we used a rabbit polyclonal from Abcam (ab#21236) for both immunoblotting and immunofluorescence. However, current batches of this antibody have failed to work for us. A mouse monoclonal antibody against Epac1 is available from Cell Signaling (#4155). This monoclonal antibody is produced by immunizing animals with a recombinant fragment of human Epac1 and works well for immunoblotting. Other antibodies for Epac are available from Abcam and Santa Cruz, but we have not tested their utility.
c. Viral Vectors
An Ad-Epac1 is available from Vector Biolabs.
d. Mouse lines
Epac1 KO mice has been described in (41). Epac2 KO mice has been described in (37).
e. Epac specific cAMP analogs
8-(4-chlorophenylthio)-2’-O-methyl-cAMP is available from Biolog Life Science Institute, Bremen, Germany.
4. References
- Borland G, Smith BO, Yarwood SJ. EPAC proteins transduce diverse cellular actions of cAMP. Brit J Pharmacol., 158: 70-86, 2009. PMID: 19210747
- Bos JL. Epac proteins: multi-purpose cAMP targets. Trend Biochem Sci., 31: 680-686, 2006. PMID: 17084085
- Boussiotis VA, Freeman GJ, Berezovskaya A, Barber DL, Nadler LM. Maintenance of human T cell anergy: blocking of IL-2 gene transcription by activated Rap1. Science, 278:124-128, 1997. PMID: 9311917
- Chaudhuri A, Husain SZ, Kolodecik TR, Grant WM, Gorelick FS. Cyclic AMP-dependent protein kinase and Epac mediate cyclic AMP responses in pancreatic acini. Am J Physiol Gastrointest Liver Physiol., 292: G1403-G1410, 2007. PMID: 17234888
- Chaudhuri A, Kolodecik TR, Gorelick FS. Effects of increased intracellular cAMP on carbachol-stimulated zymogen activation, secretion, and injury in the pancreatic acinar cell. Am J Physiol Gastrointest Liver Physiol., 288:G235-243, 2004. PMID: 15458924
- Cullere X, Shaw SK, Andersson L, Hirahashi J, Luscinskas FW, Mayadas TN. Regulation of vascular endothelial barrier function by Epac, a cAMP-activated exchange factor for Rap GTPase. Blood, 105: 1950-1955, 2005. PMID: 15374886
- Dao KK, Teigen K, Kopperud R, Hodneland E, Schwede F, Christensen AE, Martinez A, Døskeland SO. Epac1 and cAMP-dependent protein kinase holoenzyme have similar cAMP affinity, but their cAMP domains have distinct structural features and cyclic nucleotide recognition. J Biol Chem., 281:21500-11, 2006. PMID: 16728394
- de Rooij J, Zwarthkruis FJ, Verheijen MH, Cool RH, Nijman SM, Wittinghofer A, Bos JL. Epac is a Rap1 guanine-nucleotide-exchange factor directly activated by cyclic AMP. Nature, 396: 474-477, 1998. PMID: 9853756
- Dzhura I, Chepurny OG, Kelley GG, Leech CA, Roe MW, Dzhura E, Afshari P, Malik S, Rindler MJ, Xu X, Lu Y, Smrcka AV, Holz GG. Epac2-dependent mobilization of intracellular Ca2+ by glucagon-like peptide-1 receptor agonist exendin-4 is disrupted in β-cells of phospholipase C-ε knockout mice. J Physiol., 588: 4871-4889, 2010. PMID: 21041529
- Enserink JM, Christensen AE, de Rooij J, van Triest M, Schwede F, Genieser HG, Døskeland SO, Blank JL, Bos JL. A novel Epac-specific cAMP analogue demonstrates independent regulation of Rap1 and ERK. Nat Cell Bio., 11: 901-906, 2002. PMID: 12402047
- Fujimoto K, Shibasaki T, Yokoi N, Kashima Y, Matsumoto M, Sasaki T, Tajima N, Iwanaga T, Seino S. Piccolo, a Ca2+ sensor in pancreatic beta-cells. Involvement of cAMP-GEFII.Rim2. Piccolo complex in cAMP-dependent exocytosis. J Biol Chem., 277: 50497-50502, 2002. PMID: 12401793
- Fujita T, Meguro T, Fukuyama R, Nakamuta H, Koida M. New signaling pathway for parathyroid hormone and cyclic AMP action on extracellular-regulated kinase and cell proliferation in bone cells. Checkpoint of modulation by cyclic AMP. J Biol Chem. 277:22191-22200, 2002. PMID:11956184
- Fukuhara S, Sakurai A, Sano H, Yamagishi A, Somekawa S, Takakura N, Saito Y, Kangawa K, Mochizuki N. Cyclic AMP potentiates vascular endothelial cadherin-mediated cell-cell contact to enhance endothelial barrier function through an Epac-Rap1 signaling pathway. Mol Cell Biol., 25: 136-146, 2005. PMID: 15601837
- Gekel I, Neher E. Application of an Epac activator enhances neurotransmitter release at excitatory central synapses. J Neurosci., 28: 7991-8002, 2008. PMID: 18685024
- Gloerich M, Bos JL. Epac: Defining a New Mechanism for cAMP Action. Annu Rev Pharmacol Toxicol., 50: 355-375, 2010. PMID: 20055708
- Harper SM, Wienk H, Wechselberger RW, Bos JL, Boelens R, Rehmann H. Structural dynamics in the activation of Epac. J Biol Chem, 283, 6501-6508, 2008. PMID: 18167352
- Hochbaum D, Hong K, Barila G, Ribeiro-Neto F, Altschuler DL. Epac, in synergy with cAMP-dependent protein kinase (PKA), is required for cAMP-mediated mitogenesis. J Biol Chem., 283:4464-4468, 2008. PMID: 18063584
- Holz GG. Epac: A new cAMP-binding protein in support of glucagon-like peptide-1 receptor-mediated signal transduction in the pancreatic beta-cell. Diabetes, 53: 5-13, 2004. PMID: 14693691
- Holz GG, Kang G, Harbeck M, Roe MW, Chepurny OG. Cell physiology of cAMP sensor Epac. J Physiol., 577: 5-15. 2006. PMID: 16973695
- Kaneko M, Takahashi T. Presynaptic mechanism underlying cAMP-dependent synaptic potentiation. J Neurosci., 24: 5202-5208, 2004. PMID: 15175390
- Kang G, Joseph JW, Chepurny OG, Monaco M, Wheeler MB, Bos JL, Schwede F, Genieser HG, Holz GG. Epac-selective cAMP analog 8-pCPT-2’-O-Me-cAMP as a stimulus for Ca2+-induced Ca2+ release and exocytosis in pancreatic beta-cells. J Biol Chem., 278: 8279-8285, 2003. PMID: 12496249
- Kashima Y, Miki T, Shibasaki T, Ozaki N, Miyazaki M, Yano H, Seino S. Critical role of cAMP-GEFII-Rim2 complex in incretin-potentiated insulin secretion. J Biol Chem., 276: 46046-46053, 2001. PMID: 11598134
- Kawasaki H, Springett GM, Mochizuki N, Toki S, Nakaya M, Matsuda M, Housman DE, Graybiel AM. A family of cAMP-binding proteins that directly activate Rap1. Science, 282: 2275-2279, 1998. PMID: 9856955
- Kwan EP, Gao X, Leung YM, Gaisano HY. Activation of exchange protein directly activated by cyclic adenosine monophosphate and protein kinase A regulate common and distinct steps in promoting plasma membrane exocytic and granule-to-granule fusions in rat islet beta cells. Pancreas, 35: e45-e54, 2007. PMID: 17895835
- Leech CA, Chepurny OG, Holz GG. Epac2-dependent Rap1 activation and the control of islet insulin secretion by glucagon-like peptide-1. Vitam Horm., 84: 279-302. PMID: 21094904
- Lorenz R, Aleksic T, Wagner M, Adler G, Weber CK. The cAMP/Epac1/Rap1 pathway in pancreatic carcinoma. Pancreas, 37:102-103, 2008. PMID: 18580452 (Letter to Editor)
- Métrich M, Lucas A, Gastineau M, Samuel JL, Heymes C, Morel E, Lezoualc’h F. Epac Mediates beta-adrenergic receptor-induced cardiomyocyte hypertrophy. Circ Res., 102: 959-965, 2008. PMID: 18323524
- Morel E, Marcantoni A, Gastineau M, Birkedal R, Rochais F, Garnier A, Lompré A, Vandecasteele G, Lezoualc’h F. cAMP-binding protein Epac induces cardiomyocyte hypertrophy. Circ Res., 97: 1296-1304, 2005. PMID: 16269655 DOI: 10.1161/01.RES.0000194325.31359.86
- Oestreich EA, Malik S, Goonasekera SA, Blaxall BC, Kelley GG, Dirksen RT, Smrcka AV. Epac and phospholipase Cepsilon regulate Ca2+ release in the heart by activation of protein kinase Cepsilon and calcium-calmodulin kinase II. J Biol Chem., 284: 1514-1522, 2009. PMID: 18957419
- Oestreich EA, Wang H, Malik S, Kaproth-Joslin KA, Blaxall BC, Kelley GG, Dirksen RT, Smrcka AV. Epac-mediated activation of phospholipase C(epsilon) plays a critical role in beta-adrenergic receptor-dependent enhancement of Ca2+ mobilization in cardiac myocytes. J Biol Chem., 282: 5488-5495, 2007. PMID: 17178726
- Ozaki N, Shibasaki T, Kashima Y, Miki T, Takahashi K, Ueno H, Sunaga Y, Yano H, Matsuura Y, Iwanaga T, Takai Y, Seino S. cAMP-GEFII is a direct target of cAMP in regulated exocytosis. Nat Cell Biol., 2: 805-811, 2000. PMID: 11056535
- Rehmann H, Das J, Knipscheer P, Wittinghofer A, Bos JL. Structure of the cyclic-AMP-responsive exchange factor Epac2 in its auto-inhibited state. Nature, 439: 625-628, 2006. PMID: 16452984
- Rehmann H, Arias-Palomo E, Hadders MA, Schwede F, Llorca O, Bos JL. Structure of Epac2 in complex with a cyclic AMP analogue and RAP1B. Nature, 455: 124-127, 2008. PMID: 18660803
- Sabbatini ME, Chen X, Ernst SA, Williams JA. Rap1 activation plays a regulatory role in pancreatic amylase secretion. J Biol Chem., 283: 23884-23894, 2008. PMID: 18577515
- Seino S, Shibasaki T. PKA-dependent and PKA-independent pathways for cAMP-regulated exocytosis. Physiol Rev., 85: 1303-1342, 2005. PMID: 16183914
- Shah AU, Grant WM, Latif SU, Mannan ZM, Park AJ, Husain SZ. Cyclic AMP accelerates calcium waves in pancreatic acinar cells. Am J Physiol Gastrointest Liver Physiol., 294: G1328-1334, 2008. PMID: 18388188
- Shibasaki T, Takahashi H, Miki T, Sunaga Y, Matsumura K, Yamanaka M, Zhang C, Tamamoto A, Satoh T, Miyazaki J, Seino S. Essential role of Epac2/Rap1 signaling in regulation of insulin granule dynamics by cAMP. Proc Natl Acad Sci USA., 104: 19333-19338, 2007. PMID: 18040047
- Shimomura H, Imai A, Nashida T. Evidence for the involvement of cAMP-GEF (Epac) pathway in amylase release from the rat parotid gland. Arch Biochem Biophys, 431: 124-128, 2004. PMID: 15464734
- Shi GX, Rehmann H, Andres DA. A novel cyclic AMP-dependent Epac-Rit signaling pathway contributes to PACAP38-mediated neuronal differentiation. Mol Cell Biol, 26: 9136-9147, 2006. PMID: 17000774
- Ster J, de Bock F, Bertaso F, Abitbol K, Daniel H, Bockaert J, Fagni L. Epac mediates PACAP-dependent long-term depression in the hippocampus. J Physiol, 587: 101-113, 2009. PMID: 19001039
- Suzuki S, Yokoyama U, Abe T, Kiyonari H, Yamashita N, Kato Y, Kurotani R, Sato M, Okumura S, Ishikawa Y. Differential roles of Epac in regulating cell death in neuronal and myocardial cells. J Biol Chem., 285: 24248-24259, 2010. PMID: 20516079
- Williams JA, Yule DI. Stimulus-Secretion coupling in pancreatic acinar cells. In Physiology of the Gastrointestinal Tract, 4th Ed. Johnson LR Editor, Elsevier Academic Press, 2006 pp 1337-1370.
- Wu CY, DiJulio DH, Jacobson KL, McKnight GS, Watson EL. The contribution of AKAP5 in amylase secretion from mouse parotid acini. Am J Physiol Cell Physiol., 298: C1151-C1158, 2010. PMID: 20164376
- Zhong N, Zucker RS. cAMP acts on exchange protein activated by cAMP/cAMP-regulated guanine nucleotide exchange protein to regulate transmitter release at the crayfish neuromuscular junction. J Neurosci., 5: 208-214, 2005. PMID: 15634783